Implementing Circular Economy Principles in Structural Engineering Practice
Last year, the Intergovernmental Panel on Climate Change (IPCC) stated: “It is unequivocal that human influence has warmed the atmosphere, ocean, and land… Observed warming is driven by emissions from human activities. The scale of recent changes across the climate system as a whole are unprecedented over many centuries to many thousands of years.” Of course, these emissions are a product of global society as a whole. However, the building construction industry has been a significant emissions contributor and material user; approximately 40% of global material extraction is for housing, construction, and infrastructure. As structural engineers, we have a fantastic opportunity to be impactful leaders in reducing material use and emissions.
Decisions we make to reduce the embodied carbon in our project work can save hundreds of tons of carbon emissions each year. This is orders of magnitude more impactful than decisions we make in our personal lives – such as driving less or taking fewer flights – which can save a few tons of carbon emissions each year. This article discusses the potential to reduce greenhouse gas emissions by defining a Circular Economy in the architecture, engineering, and construction (AEC) industry, reviewing key case studies that implement Circular Economy principles, and outlining future goals of the Structural Engineering Institute (SEI) Sustainability Committee’s Circular Economy Working Group.
What is a Circular Economy?
The emissions associated with material use are primarily related to the current industry paradigm: a linear economy. A linear economy in construction can be categorized by a take-make-waste model (Figure 1), where raw materials are extracted (take) and processed into construction materials for use in our built environment (make). At the end of life, structures are demolished, and the materials are typically discarded to landfill or, more commonly, downcycled (waste). Each of these stages emits greenhouse gases: energy use for extraction, energy use and chemical reactions during processing, and fuel use in transporting materials from different locations throughout the material and construction lifecycle. This linear economy is common for construction materials such as concrete, brick, or timber, where the material is often processed into subbase or mulch with a much lower value. Metal construction materials at their end-of-life are typically recycled (a large loop economy). While this is a vast improvement over landfill, the recycling process is a large loop that still requires energy contributions for sorting, processing, and transporting before following the process of refining and producing elements again from scratch (Figure 2).
In contrast to a linear economy or large loop economy, a circular economy in construction aims to minimize waste and emissions by reducing the need for new materials and fabrication processes through tighter construction material loops (Figure 3). The primary goal is to extend the use of a structure through renovation, refurbishment, and re-purposing – extending the length of the use phase. Where this is not possible, and a structure comes to its end of life, a circular economy model allows for the repair, reconditioning, reuse, and refurbishing of existing elements, and designing new structures and components for longer lifespans and better reusability and repair from the outset. These changes from a linear to a circular economy model can reduce the emissions associated with material extraction, processing, and transport. These changes can also provide economic benefits if realized, particularly in a world where energy and fuel costs are increasing and volatile. As the Circular Economy Working Group for the SEI’s Sustainability Committee, we use the following definition:
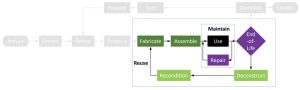
A circular economy is one that keeps or elevates structural products, components, and materials at their highest utility and value across multiple life cycles within the building stock. Through maintaining structural elements at their highest utility, we can: reduce greenhouse gas emissions associated with manufacturing new structural elements; minimize impact on natural environments caused by new material extraction; and, for biogenic materials such as wood, continue to sequester embodied carbon for longer.
This circular economy definition focuses on the reuse of individual products, components, and materials, as opposed to the reuse in place of complete systems like buildings or bridges. The refurbishment, adaptive reuse, and retrofit of existing structures – where components are maintained in place – is an essential part of implementing circular economy principles in the AEC industry and is well covered by other groups, such as the Structural Condition Assessment and Rehabilitation of Buildings SEI Codes and Standards Activities Division committee. In most cases, maintaining a structure in its current use with most of its materials in place leads to the smallest greenhouse gas emissions. However, in scenarios where maintaining an existing structure in place is impractical, circular economy principles applied to a structure’s individual components are important.
Implementing a circular economy in construction is a very achievable goal. Indeed, for most of the history of construction, dismantling derelict structures to reuse the components in new buildings was not unusual. This stretches from St Peter’s Basilica in Rome, Italy, which was partially constructed using stone salvaged from the nearby Colosseum, up to early 1900s construction in NYC and Chicago, where buildings were routinely manually disassembled, with their bricks, timber joists, and structural steel parts stockpiled and sold for reuse in new structures. In previous contexts, using available salvaged structural materials – instead of paying to manufacture and transport new materials – was part of standard practice and incentivized by lower structural material costs for reused elements. However, demolition practice began to change at the end of the Gilded Age when the speed of building removal was emphasized in place of opportunities for salvage. Examples include the demolition of the 20-story Gillender building in NYC in 1910. By the 1950s, the methodical manual labor that allowed materials to be processed for reuse was replaced with heavy machinery (such as wrecking balls and bulldozers), reducing the opportunities for reuse. The widespread mechanized demolition ushered in by the Housing Act of 1949 and the Federal Aid Highway Act of 1956 could be seen as marking the transition from the previous period of a more circular economy in construction to that of a linear one.
These changes in demolition practice were accompanied by changes in the AEC industry related to the development of building codes and construction material specifications. In 1922, before these changes took place, the Building Code of NYC discussed methods for reusing old bricks in new construction and explicitly noted that old steel and wrought iron shapes could be reused. The American Engineering Standards Committee was only founded in 1918 and had a limited scope of influence. Today, ASTM standards are diverse and numerous, and building codes typically specify new material that meets these standards. The importance and value of building codes and specifications to the industry and society are evident. Still, one side-effect of the codification was the preference for certification that controls new industrialized manufacturing processes instead of processes that enable reconditioning and recertifying existing materials. This can become a barrier to re-implementing circular economy principles in the industry today. Extensive material investigation and testing are required to reuse existing materials that lack documentation, such as steel mill certification, to meet standard specifications such as ASTM A500 for chemical composition and material strengths. Many changes away from the status quo linear economy model of material specification, element design, and new material procurement bring a similar potential for increased uncertainty and associated increases in cost.
While barriers to re-implementing a circular economy exist, they are not insurmountable. Structural engineers making design decisions on element sizes and material specifications have a fantastic opportunity to explore circular economy principles in our work and realize some of the carbon emission reductions available to us. In addition to the historical precedent and environmental motivation, the global economy may assist in this new transition from a linear economy back to our previous circular one. Since 2020, the pressures on supply chains and transport logistics have led to high and unpredictable material prices. As a result, the ratio between material and labor costs is changing. In partnership with a global imperative to reduce greenhouse gas emissions, this economic pressure could lead to a construction ecosystem where a linear economy reliant on a steady supply of new material is not desirable or economically viable.
Fortunately, the principles of circular construction are still at the heart of our profession. Engineers work to a client’s brief and within project constraints to solve problems; our role is essentially as a resource manager, balancing costs, time, and material use as best we can while fulfilling the project. To address the climate emergency, we can re-prioritize these resources, with a renewed focus on materials – and their associated greenhouse gas emissions – in addition to time and cost. As engineers, we can continue to react to economic pressures, but we can also proactively be involved in discussions to implement changes in legislation and practice to focus more on material lifecycle management and specification. For example, we could design for sustainability as well as serviceability and strength. The structural engineering industry is already proactively addressing our impact on greenhouse gas emissions through initiatives such as SE2050; however, there are further opportunities for us to specifically address material life cycles through implementing circular economy principles.
Case Studies
While circular construction practices have become less common, they are not unheard of. Existing case studies can be valuable examples of the potential for material reuse and design for deconstruction. In addition, they can be used to demonstrate to design teams and clients that perceived barriers to reuse or design for deconstruction can be overcome. There have been several projects completed in the last 25 years that successfully demonstrate either (1) the reuse of materials in new construction or (2) the design for deconstruction (DfD) of new structures to facilitate future material reuse at the structure’s anticipated end-of-life. The following highlight a few case study examples for different primary construction materials extracted from an expanding database of benchmark circular economy projects currently being collected by the Circular Economy Working Group. You can find additional information on many of these examples in the list of references below.
First, case studies that reuse existing material in new construction and facilitated by construction techniques with discrete elements that can be connected, disconnected, and reconfigured. This typically prefers frame or panel construction from steel, timber, or precast concrete instead of monolithic construction from cast-in-place concrete.
The Mountain Equipment Co-op in Ottawa, Ontario, is a commercial building constructed in 2000. The steel structure on the site was deconstructed, and components were reused to construct the new steel frame. On the scale of local infrastructure instead of buildings, Muskingum County Engineers Office in Zanesville, Ohio, has reused existing steel beams from previous projects to construct seven new bridges between 16 feet and 55 feet between 2007 and 2015. Concerted industry efforts in the United Kingdom have led to a recent drive to normalize the use of reused steel in new construction, with the publication of the practical design guide Structural Steel Reuse by the Steel Construction Institute in partnership with Cleveland Steel and Tubes Ltd.
As demonstrated by the Eglisau bridge, timber elements have been reused successfully for centuries. A successful example of timber reuse has been the Wilson Farm barn in Lexington, Massachusetts, completed in 1996. This incorporated the use of timber from former U.S. army facilities and commercial factory buildings, among other locations. An in-depth body of research has been completed by the U.S. Department of Agriculture Forest Products Lab in Madison, Wisconsin, to propose methods to enable salvaged wood to be reused, although commercial barriers remain to reuse. Recent research by the InFutUReWood group explored the obstacles to salvaged timber reuse and focused on reusing material in laminated engineered wood products.
As a heavy frame or panel material, precast concrete has not featured in material reuse projects as frequently as steel or cast-in-place concrete. However, some successful case studies have been conducted, such as the Plattenpalast art space constructed in Berlin, Germany, in 2009. The design utilized standardized concrete panels from a standard construction type and reconfigured them with new connections to form a new structure. In addition, recent research at École Polytechnique Federale de Lausanne proposed the manufacture of new precast concrete blocks by cutting them from existing cast-in-place concrete elements and connecting them instead of reusing previous precast concrete elements. Considering the quantity of cast-in-place concrete in our built environment, this seems a challenging but potentially high-impact approach.
Secondly, new construction that is designed for future deconstruction can be considered. In contrast to material reuse in construction, design for deconstruction projects plan for potential future material reuse while still utilizing conventional material workflows for the initial construction. This removes a number of the certification and supply-chain obstacles to material reuse. However, it relies on future favorable deconstruction and reuse practices that take advantage of the initial design and delays the opportunity to benefit from the circular economy until the building’s end of life instead of at the structure’s outset.
A recent exciting large-scale example of steel design for deconstruction is Stadium 974, one of the soccer stadiums constructed in Qatar for the 2022 World Cup. This stadium is envisaged as a bolted modular steel frame that can be easily dismantled, transported to alternative locations, and reconfigured into a series of different smaller stadium-style structures.
The use of precast concrete elements in design for deconstruction projects benefits from commercial development in embedded bolted connection systems. An interesting innovation for Circle House in Aarhus, Denmark, is the use of a lime mortar to seal around the metal hardware fixings instead of conventional cement grouts. Using a lime mortar allows for removing the sealant for deconstruction as opposed to the more permanent cement alternative.
Timber construction designed for deconstruction requires a focus on discrete reversible connections. These can vary from traditional bearing joist hangers and column head interfaces, removable fasteners such as screws, or interlocking carpentry solutions from Japanese or Western traditions, as demonstrated in much of Kengo Kuma’s work. Modern mass timber frame construction, such as the Bullitt Center in Seattle, Washington, utilizes metal plate connectors screwed between columns and beams to allow for potential deconstruction and element reuse with limited damage.
A Call to Arms
Existing case studies that implement circular economy principles in construction are an essential basis for sharing lessons from successful projects – located in North America or other regions. These case studies can teach us how existing barriers to the circular economy model can be overcome, whether through demonstrating the material recertification, laboratory testing and warranties, or showcasing innovative technical design solutions. However, the SEI Sustainability Committee Circular Economy Working Group would like to do more to foster a supportive base to allow all design professionals to advocate and implement circular construction in their work. The Group believes this requires some additional work.
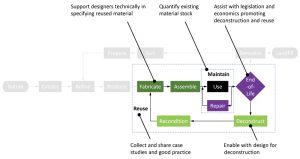
The Circular Economy Working Group intends to focus its work at the level of structural components (i.e., beams, columns, truss elements) instead of complete systems (i.e., buildings, bridges) through the pursuit of design for deconstruction and element reuse as opposed to renovation or refurbishment, or Design for Adaptability. The objectives of the Circular Economy Working Group (Figure 4) are to:
- Quantify the structural components within our existing built environment, which could become available for future reuse, focusing on common and repetitive structural typologies to guide circular economy efforts.
- Maximize reuse of existing construction materials, promote deconstruction over demolition, and facilitate design with reused materials.
- Facilitate future deconstruction of structures by promoting Design for Deconstruction methods, and good-practice project specifications.
- Assist with introducing a legislative framework for promoting Circular Economy practice in the construction industry through proposed policy and local ordinances.
- Collect and share case studies and good practices from previous projects that have been completed which align with Circular Economy principles.
The need to change from a linear economy in construction to a circular economy is clear and pressing – a change in how structural engineers design and specify materials in our projects can significantly reduce the greenhouse gas emissions associated with our work. Unfortunately, the AEC industry changed in the latter part of the 20th century to a more linear economy which has accumulated practices that are now barriers to implementing circular economy principles easily and cost-efficiently. But, crucially, the barriers to this change are not insurmountable, and, as structural engineers, it is our responsibility to do more to facilitate the transition back to a circular economy. The Working Group looks forward to implementing this change together.
If you have information to share regarding any of these five objectives, please contact the SEI Sustainability Committee Circular Economy Working Group at the author’s email address.■
References
IPCC Sixth Assessment Report – Climate Change 2021: The Physical Science Basis www.ipcc.ch/report/ar6/wg1
UNEP 2020 Global Status Report for Buildings and Construction https://wedocs.unep.org/bitstream/handle/20.500.11822/34572/GSR_ES.pdf?sequence=3&isAllowed=y
Circle Economy – The Circularity Gap Report 2018
https://pacecircular.org/sites/default/files/2020-01/Circularity%20Gap%20Report%202018_0.pdf
Carbon Footprint Carbon Calculator www.carbonfootprint.com/calculator.aspx
IstructE 2022 – How to Calculate Embodied Carbon www.istructe.org/resources/guidance/how-to-calculate-embodied-carbon
CIRCuIT – About Circular Construction www.circuit-project.eu/about-circular-construction
SEI Sustainability Working Groups https://seisustainability.org/working-groups
The Engineering Behind Saint Peter’s Basilica https://engineeringrome.org/the-engineering-behind-saint-peters-basilica
OLD HOUSES HELP MAKE NEW ONES: Second-hand Material Is Often More Serviceable Than a Fresh Supply. Detroit Free Press (1858-1922); Jan 15, 1911
Breaking Records in House Wrecking, The New York Times (New York, New York) – Fri, Jun 3, 1910, Page 2
“Rubble: Unearthing the History of Demolition,” Jeff Byles, 2005, Broadway Books, New York
Building Code of the City of New York,1922, Bureau of Buildings, New York
STRUCTURE, February 2022 – Calling on Structural Engineers to Design for Sustainability www.structuremag.org/?p=19791
Mountain Equipment Coop, Ottawa / www.canadianarchitect.com/designing-for-disassembly
STRUCTURE, February 2016 – Sustainable Bridge Building Practices www.structuremag.org/?p=9558
SCI P427 – Structural Steel Reuse https://steel-sci.com/assets/downloads/steel-reuse-event-8th-october-2019/SCI_P427.pdf
USDA FPL – Framing Lumber from Building Removal www.fpl.fs.fed.us/documnts/pdf2013/fpl_2013_falk001.pdf
InFutURe Wood – Design for deconstruction and reuse of timber structures www.infuturewood.info/wp-content/uploads/2021/02/InFutURe-Wood-Report-D2.1f.pdf
InFutURe Wood – Product Design Using Recovered Timber www.infuturewood.info/project/workpackage-3-product-design-of-recovered-timber
Plattenpalast www.reduce-reuse-recycle.info/Projekt_3_0_id_83.html
RE:CRETE footbridge www.epfl.ch/labs/sxl/index-html/research/reuse-of-concrete
Stadium 974 www.sbp.de/en/project/stadium-974
Circle House https://bit.ly/3REZJ3e
Interlocking Timber Joint in Kengo Kuma’s Work https://issuu.com/xinsuan.selectedworks/docs/interlocking-timber-joint-in-kengo-