HCM can effectively reduce the impact of stressors and stressing events.
We’ll start with some definitions. “Resiliency” has become a popular buzzword in design circles, but it often has various meanings depending on the user and the target audience. For this article, we will use “resiliency” to define the human characteristic of the ability to return to normalcy after a significant stressful event, or the ability to bounce back to normalcy from a significant stressful event. Some stressors can permanently change the definition of normalcy, such as loss of life that is not something from which one can bounce back. Normalcy must be redefined in such instances.
A stressful event can be anything of sufficient magnitude, time, or both, interrupting a person’s normal daily routine, family, neighborhood, community, country, or even broader humanitarian base. As an example of a significant stressor, consider Covid-19. It impacted individuals, families, neighborhoods, communities, and many entire countries and is considered an example of a worldwide stressor. To some extent, it remains as such. All aspects of everyone’s lives have not returned to the pre-Covid-19 normal status. Some things will remain permanently redefined regarding normalcy. In terms of the audience, this article, focuses on building-related stressors and building responses to those stressors in relation to human resiliency.
Some common events that are significant stressors that impact individuals due to their consequential effects on structures are: high winds; tornadoes; hurricanes; tidal surges; tsunamis; wildfires; ground motion; earthquakes; explosions; civil unrest, and even terrorism. There are others. Natural occurring stressors and even human-caused stressors, impact individuals by impacting their ability to function normally. If a significant stressor destroys one’s home, and food is needed, but the grocery stores have all been destroyed, no food can be acquired. That is a significant stressor, and the longer the lack of food persists, the worse of a stressor the event becomes. If medical attention is needed and the hospitals, emergency rooms, and urgent care facilities are all destroyed, that is a significant stressor. If communication necessary for survival is disrupted, that is a significant stressor and worsens with time.
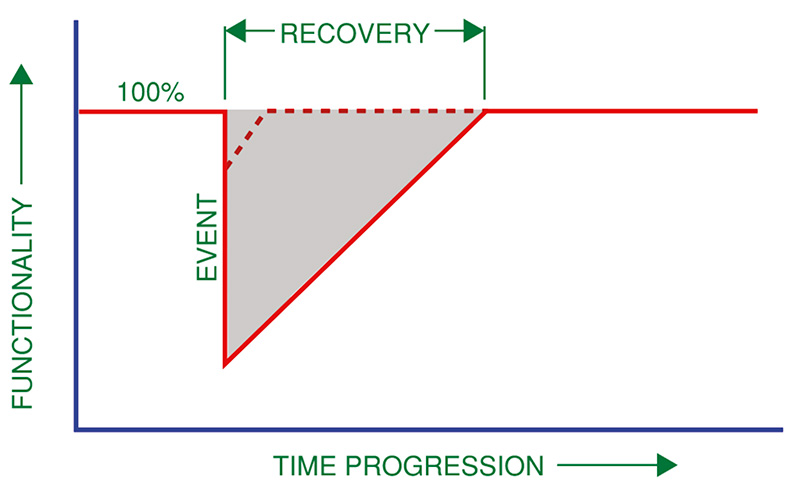
The impact of stressors and resiliency can be represented by a graph that identifies the stressor disruption to normalcy, and the recovery period, called the resiliency triangle, as in Figure 1.
The dashed red line represents a minor disruption or stressor, whereas the solid red line is a significant disruption or stressor.
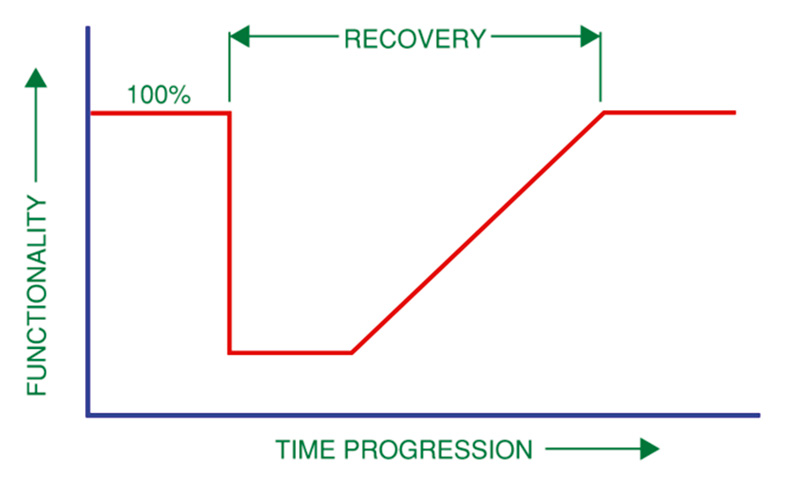
When a stressor or disruption occurs, that event reduces the ability to function normally by some amount – the vertical drop in functionality. After the stressful event, the return to normalcy can be quick or prolonged. Suppose goods and materials need to be acquired, and there is a disruption in the supply chain for needed supplies or materials. In that case, the duration of the stressor can be extended, which may actually amplify and/or acerbate the stressor. Initial injuries experienced during the stressor can become infected due to lack of proper medical attention, which can reduce one’s ability to recover, so the impact of the event (degree of reduction of normalcy and duration of recovery) is increased. See Figure 2, which represents a large stressor that takes an extended time to mobilize and restore normalcy. This becomes a resiliency trapezoid (still referred to as a resiliency triangle).
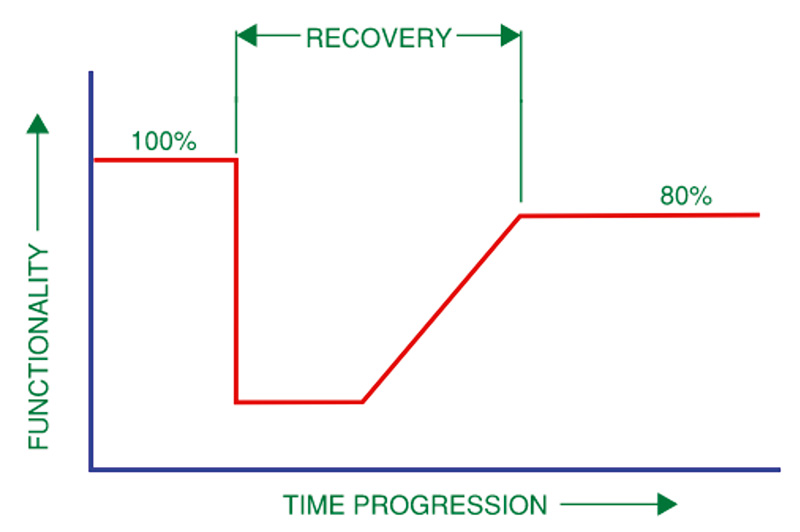
Figure 3 represents a scenario where a return to normalcy is not possible, and a new level of normalcy is defined, such as permanent loss of functionality – loss of limb, loss of life, building collapse, etc.
Natural occurring stressors and even human-caused stressors, tend to be random and often unpredictable. Some events broadcast themselves days in advance – hurricanes, while others only make themselves known minutes or hours in advance – tornadoes. Earthquakes, wildfires, civil unrest, and terrorism are often spontaneous and unforeseen events without much warning. How can one design for those, one might ask?
In most cases, designers generally have a performance history for recurring events. Academic research can provide enlightenment. Worst-case scenarios can be investigated. All of these scenarios are used to inform codes that, in turn, require or prescribe minimum requirements to be used to design facilities/buildings/structures that are intended to preserve life. There is recognition in the model building codes for what has been deemed performance design, which describes the anticipated functionality of a facility after a given design event (stressor). Functionally, model building codes have always primarily focused on preserving the lives of the building occupants, not the preservation of property; collapse avoidance and preservation of building function were the goals. Model building codes have recognized for some time that the functionality of some buildings is extremely valuable in post-stressor periods. This design concept crosses the threshold of designing for collapse prevention to designing for sustained performance.
Preserving the functionality of a structure is of major importance when talking about services that are needed after a significant stressor. Hospitals, police stations, telecommunications equipment, governmental buildings, command and control centers, etc. The challenge is defining the correct design event because naturally occurring events do not have predictable repeatability; they have probabilities of recurrence for a given magnitude. Any given design event has at least some probability of being exceeded in any given period. A ”100-year-storm”, for instance, can occur several times in any given year, and data is then used to redefine what is a “100-year-storm”. Historical data is continually being analyzed to inform future design.
In many cases, the typical way to enhance performance or increase durability – to give the nod to the likelihood that any given design event can be exceeded – is to design for higher design loads, provide continuity and connectivity to the load paths in the building via special detailing, add redundancy (more load paths), add ductility, and use higher strength materials. Making more robust force-resisting systems and tying all the parts and pieces of the building together to avoid partial or cascading collapse is the goal. These concepts increase the durability of the building – the ability of the building to withstand the assigned or prescribed design forces or even forces that exceed those assigned or prescribed. One can easily equate increased durability to enhanced resiliency. Suppose a structure has sufficient capacity to withstand prescribed or assigned forces with little or no damage. In that case, individuals needing to use those facilities can more easily and quickly return to normalcy when the facilities remain functional. The resiliency of those individuals has been enhanced. Structural durability equates to enhanced resiliency.
So, how does all this relate to Hollow Clay Masonry (HCM)? Those familiar with structural masonry probably deal primarily with Concrete Masonry Units (CMU) and probably design for units that comply with the minimum design strength required by ASTM C90 – Standard Specification for Loadbearing Concrete Masonry Units, which requires a minimum net compressive strength of 2,000 psi (13.8 MPa). This, in turn, equates to the masonry wall assembly design strength (f’m) of 2,000 psi (13.8 MPa) per TMS 602-16, or TMS 602-22, assuming the use of either Type M or Type S mortar and grout strength (f’g) equal to the assembly strength of 2,000 psi (13.8 MPa). Some CMU designers use f’m of 2,500 psi (17.3 MPa), as most CMU manufacturers can easily make units with a minimum net compressive strength of 3,250 psi (22.4 MPa) that is needed to achieve f’m = 2,500 psi (17.3 MPa).
If one wants a more robust structure, one chooses higher-strength materials. This is a well-known and historically proven axiom. HCM is routinely made with gross compressive strength far in excess of the ASTM C652 – Standard Specification for Hollow Brick (Hollow Masonry Units Made From Clay or Shale) minimum requirements; 3,000 psi (20.7 MPa), which works out to around 6,000 psi (41.4 MPa) for the net compressive strength. Typical net compressive strengths for modern fired clay (and shale) structural masonry units range from 8,000 psi (55.2 MPa) upwards of 20,000 psi (138 MPa), depending on the clay (and shale) materials, the material mix, and the firing temperature(s), used by brick manufacturers. This high HCM unit compressive strengths generally produce wall assembly design strengths (f’m) of 3,500 psi (24.2 MPa) to 4,000 psi (27.6 MPa) using Type M or Type S mortar and using grout strength (f’g) equal to the wall assembly design strength (f’m) as per TMS 602-16 or TMS 602-22.
Similar to the steel design concept of using ASTM A992 Gr 50 rather than ASTM A36 steel to increase capacity, using HCM to provide enhanced capacity, as compared to CMU, is as easy as using f’m = 3,500 psi (24.2 MPa) or 4,000 psi (27.6 MPa) rather than 2,000 psi (13.8 MPa) or 2,500 psi (17.3 MPa). All the same masonry design philosophies and masonry design equations are used for the two materials.
Stiffness (modulus of elasticity) of structural masonry, as prescribed by TMS 402-16 and TMS 602-22, is a function of the wall assembly design strength, f’m. For CMU EmCMU = 900 x f’m. For HCM, EmHCM = 700 x f’m. That would lead one to believe that HCM buildings are more flexible than CMU. However, because the typical or common wall assembly design strengths (f’m) for HCM are around 40% to 60% higher than for typical CMU, the stiffness of the two systems is very similar. With similar stiffness, similar seismic forces are delivered to the structure, whether it be HCM or CMU.
For the following wall shear capacity and axial load capacity comparisons, common structural masonry wall assembly design strengths (f’m) of 4000 psi (27.6 MPa) for HCM and 2500 psi (17.3 MPa) for CMU will be evaluated.
SHEAR CAPACITY
The TMS 402-16 (or TMS 402-22) shear capacity equations for Allowable Stress Design (ASD) (for other than special reinforced shear walls) and Strength Design (SD) are as follows:
ASD: Fvm = (1/2) x [(4.0-1.75(M/Vd))√f’m] + axial load component Eqn. 8-26; 8-23
SD: Vnm = [(4.0-1.75(Mu/Vudv))Anv√f’m] + axial load component Eqn. 9-20; 9-18
For a given wall geometry: (4.0-1.75(M/Vd)) and (4.0-1.75(Mu/Vudv)) are the same for CMU and HCM in ASD and SD, respectively; the axial component is the same for CMU and HCM in ASD and SD, respectively. The two equations simplify the comparison of √f’mCMU vs. √f’mRHCM . In both ASD and SD, for a given shear wall geometry (without reinforcing contribution), the HCM wall has 26.5 % higher shear capacity/strength than the same wall configuration in CMU. Reinforcing will add the same augmented strength to both wall types, but the HCM wall will generate higher capacity/strength, and require shorter lap lengths, saving some steel weight. HCM can, in many instances, reduce the shear reinforcing required to attain the same wall capacity/strength, which reduces cost.
The image below is a comparison of the unreinforced grouted wall capacity contribution of HCM and CMU in ASD for reinforced masonry shear walls.
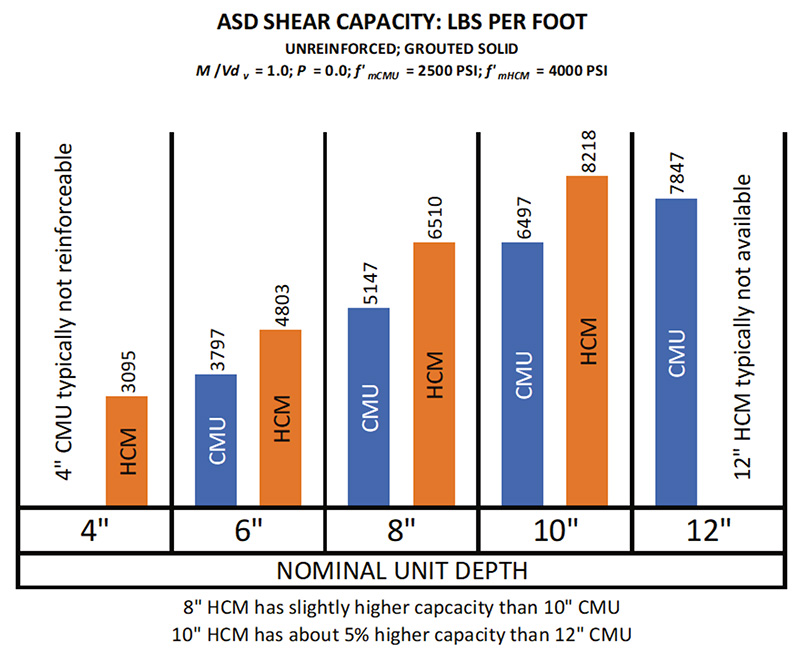
For higher shear demand, HCM walls may be able to avoid increasing the wall thickness to attain the needed capacity, as compared to CMU. Of course, reinforcing increases the strength/capacity, but less reinforcing is possible using HCM to reach the same degree of capacity.
AXIAL CAPACITY:
The TMS 402-16 (or TMS 402-22) axial capacity equations for ASD and SD, for h/r ≤ 99, are as follows:
ASD: Pa = (0.25†f’mAn + 0.65AstFs)[slenderness reduction] Eqn. 8-18/19; 8-16/17
SD: Pn = 0.80[0.80f’m(An-Ast) + fyAst][slenderness reduction] Eqn. 9-15/16; 9-9/10
†: The multiplier of 0.25 in TMS 402-16 changes to 0.30 in TMS 402-22
For a given wall configuration: An, Ast, Fs, and fy are the same for CMU and RHCM in ASD and SD, respectively; the slenderness reduction is the same for CMU and HCM in ASD and SD, respectively; and the multipliers of 0.25 [0.30], 0.8 and 0.8 are the same for CMU and HCM in ASD and SD, respectively. The capacity/strength equations simplify down to the comparison of vs. In both ASD and SD, for a given wall configuration (without reinforcing contribution), the HCM wall has 60% higher axial strength than the same configuration in a CMU wall. This higher capacity can, in many instances, reduce the reinforcing required to get the same wall performance. For a given wall height, the HCM wall can carry more load. The HCM wall can generally span farther vertically for a given wall load, or a thinner wall may be possible. However, the slenderness reduction can become more significant for those cases.
EleMasonry software, by Ensoltech, was used to produce the above interaction curves. Of note: the area enclosed by the HCM curve for this wall is more than twice the area enclosed by the CMU curve. Also of note, for lightly loaded walls (low demand: ≈ 25% of the CMU axial capacity), there is little advantage of HCM over CMU as the curves are almost coincidental. The great advantage of the higher strength achievable with HCM is illustrated by the dual interaction diagram. When axial loads exceed roughly half the axial capacity of the CMU, then HCM has much more capacity available, up to roughly 60% higher than the axial capacity of the CMU.
For any given design in CMU, swapping HCM for CMU while maintaining the same wall thicknesses and reinforcing generates an immediate increase in capacity and durability, which increases the ability to resist induced forces, which equates to the potential for less damage, less repair, and faster recovery, culminating in improved resiliency.
In addition to the higher strength that can be provided by common HCM for a given wall geometry, HCM, like CMU, has proven fire-resistance ratings, up to 4-hour fire-resistive ratings for 8-inch-thick filled walls. Grouted HCM walls, 6-inch-thick and 8-inch-thick, by one manufacturer have passed the FEMA projectile test for tornado wind speeds up to 250 mph. HCM has been used as the exterior reinforced façade on a Federal Court Building in compliance with General Services Administration blast resistant criteria. Lastly, special profile HCM produced by one manufacturer has passed a producer-specified 17-protocol ballistic resistance test including .22 caliber, .223 caliber, 9mm, .308 caliber (including armor piercing), .30-06 (including armor piercing), .357 caliber magnum, 44 caliber magnum, and .50 caliber shots, including 12-ga shotgun – slugs and buckshot, all fired from a distance of 15 feet.
When used in at least modest amounts in buildings, structural masonry – HCM and CMU – routinely produce facilities that embody high durability and increased redundancy. The masonry elements help resist the spread of fire, with 8” walls able to achieve up to 4-hour fire-resistive ratings that function 100% of the time at no extra cost. Less fire spread can mean reduced property loss and reduced loss of life. Both reductions enhance resiliency. The structural masonry walls have high projectile and ballistic resistance, with some HCM systems able to resist extremely high-energy munitions.
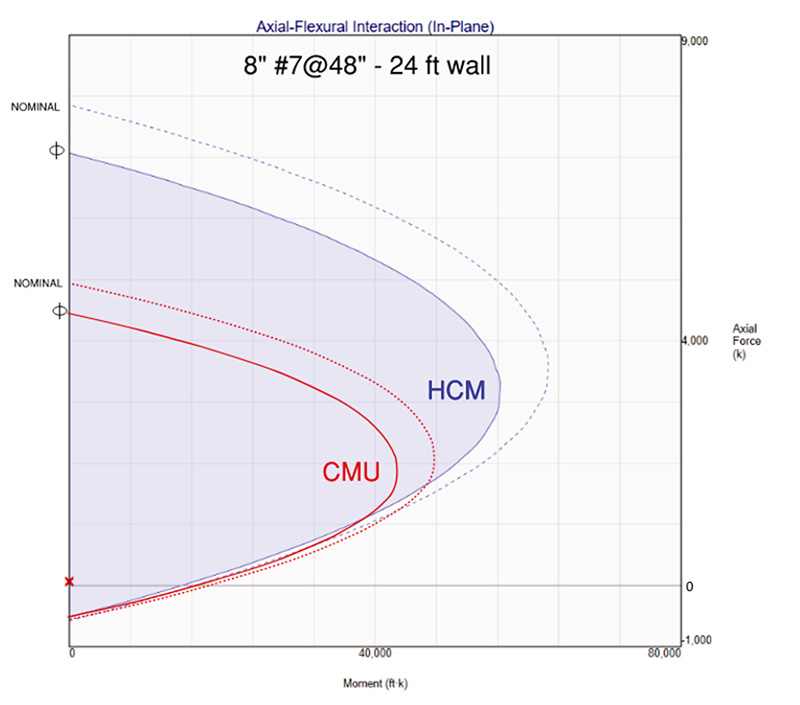
HCM has a highly durable surface that is color-fast, does not fade, and does not need routine painting or touchup as maintenance, which reduces downtime for repairs after certain stressing events, like flooding, projectile impact, or abuse. Reduced downtime increases resiliency. In 2017, Hurricane Harvey hit Houston in late August 2017, just before schools were to open in the fall. Roughly 30 school campuses could not open on time due to flooding damage. At least nine campuses were so badly damaged that new locations needed to be found. Portions of interior gypsum board walls that were underwater had to be removed, and mold mitigation methods were employed; gypsum board had to be replaced and painted to match existing walls. If the interior walls had been made of structural masonry, as in many schools, once the water was pumped out, the walls could have been pressure washed and dried quickly and schools opened without delay to the school year. Resilience of the school district employees and students could have been enhanced by using structural masonry in those schools for quicker recovery from the flooding.
HCM can provide higher capacity/strength using commonly available materials for any given wall geometry. HCM can be used in common sizes to produce more robust structures that are more durable than other common masonry building materials. More durable facilities can withstand higher loads, incur less damage, and reduce repair time and costs. In other words, HCM can effectively reduce the impact of stressors and stressing events and shrink the resiliency triangle for faster recovery, which equates to enhanced resiliency.■